Basic research nourishes the growth of photonic
metamaterials and plasmonics, while the desires of the consumer
result in laser TV and higher-capacity optical disks. At the center
of it all is the photonics industry, making things
happen.
Going to a photonics conference to gawk at the technology can be
a rewarding experience. There one can see, in the technical
sessions, some rather stunning achievements. While no physical laws
are violated as leading-edge researchers discover new ways to
exploit light in science and engineering, they certainly seem to
come close to bending the rules.
Spending time on the exhibit floor can be just as satisfying-that
is where one finds the practical results of all this research. In
fact, the interaction between research and commercialization is not
a one-way street. Anyone who spends time in the technical sessions
will discover presentations given by researchers from photonics
companies worldwide, both large and small. This technology feeds
back to the universities, perhaps through a post-session
conversation between interested parties or a later formal
collaboration. In addition, the latest and greatest optical
instruments seen on the exhibit floor become the building blocks for
future research setups.
Sometimes, the results of this research are not found on the
exhibit floor of a photonics show. Instead, they find their way into
your automobile (advanced lighting, fiber-optic communications),
your computer (optical data storage and, someday soon, optical data
interconnects), or your pocket (digital-camera sensors, novel lenses
for cell phones and cameras). In this way the photonics industry,
already many billions of dollars in size, is edging closer to
becoming a behemoth the likes of the electronics industry.
Bringing practical optical interconnects for computers
(board-to-board, chip-to-chip, and perhaps intrachip) and other
integrated photonics a step closer to reality, a group of
researchers at Intel and the University of California-Santa Barbara
(both of Santa Barbara, CA) fabricated electrically injected indium
phosphide (InP) 1550-nm-emitting diode lasers on silicon, while
researchers at Ghent University (Ghent, Belgium) and the Technical
University Eindhoven (Eindhoven, The Netherlands) independently
created the same sort of device, but used a different approach (see
“Two groups create electrically injected hybrid lasers on silicon,”
www.laserfocusworld.com/articles/274700).
In the Intel version, an InP light source is bonded to a
silicon-on-insulator (SOI) waveguide with a mere-nanometers-thick
oxide layer serving as the glue via a high-temperature bonding
process; the SOI becomes the laser cavity (SOI is an easy-to-create,
optically transparent layer well-suited to integrated photonics). In
contrast, the Belgian/Dutch device uses benzocyclobutene, a polymer,
as the adhesive, with a complete InP laser coupling its light into a
layer of SOI via a polymer inverted-taper waveguide (see Fig. 1).
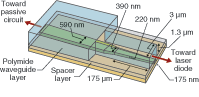
FIGURE 1. An adiabatic inverted-taper structure
couples light from an InP laser diode (not shown) from a
polyimide waveguide layer into an SOI waveguide (green) on
silicon. |
The European group also created similar devices with slightly
different geometry that served as photodetectors. Shortening the InP
section from the laser’s 500 µm to 50 µm for the detector, the
researchers measured a responsivity of 0.23 A/W at 1555 nm.1
In another yield from its continuing push into silicon photonics,
Intel has created a high-power monolithic integrated Raman
ring-cavity silicon laser that emits up to 30 mW at a 686 nm
wavelength.2 Because the
laser’s structure can be fabricated entirely by processes akin to
conventional computer-chip fabrication (as opposed to the InP hybrid
lasers, which must be manipulated into place and then bonded onto
SOI), this sort of laser is potentially very low in cost and can be
highly integrated with other silicon photonics. The laser is,
however, optically pumped (at 1550 nm). Cascading these lasers,
each of which lengthens the wavelength of its pump light, can
potentially result in a room-temperature mid-IR semiconductor laser.
Research into metamaterials proceeds apace (see “Meta 2006
highlights random, periodic optical metamaterials,” www.laserfocusworld.com/articles/266371), with one
result in particular capturing the attention of the popular press:
cloaking for invisibility. In the scheme, developed by John Pendry
of Imperial College (London, England) and others, a metamaterial
structure surrounds an object, redirecting any light striking the
metamaterial so that it swerves around the object, then exits the
cloak on the other side along its original path, making the object
appear not to exist.3
One problem is what the popular press has done with this
idea-like describing fanciful devices such as mantles that hide cars
or people. In reality, scientists take pains to make sure that a
metamaterial is mechanically stable to dimensions much smaller than
the relevant wavelength of electromagnetic radiation; this
requirement, along with metamaterials’ significant attenuation of
light, means that optical-metamaterial-based invisibility cloaks
millions of wavelengths in extent (in other words, people-hiders)
are impractical.
A cloak of invisibility has, however, been demonstrated at
microwave frequencies.4
David Schurig and his colleagues at Duke University (Durham, NC),
Imperial College (London, England), and SensorMetrix (San Diego, CA)
rely on a “transformation-based” cloak design (one in which
Maxwell’s equations undergo coordinate transformations) to channel
8.5 GHz (3.6 mm) microwaves around a 50‑mm-diameter copper
cylinder. The cylindrical metamaterial structure approximates a 2-D
(planar) cloaking device, rather than a full 3-D device; has a
lattice spacing of about 3.3 mm, and results in partial invisibility
(see Fig. 2). The results are of interest for both civilian
(cell-phone transmission) and military stealth use.
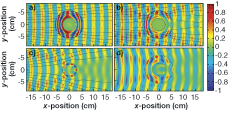
FIGURE 2. A simulation of a microwave cloak with
ideal material properties shows a high degree of cloaking (a),
while a simulation based on material properties of the actual
structure provides less cloaking (b). Experimental measurement
of microwave propagation around a bare copper cylinder reveals
a shadow (c); measurement of the cloaked cylinder shows that
the shadow is reduced, although not eliminated (d).
|
While they once held the position of most exotic laboratory
optical material (a spot now occupied by metamaterials, or perhaps
surface-plasmon structures), photonic crystals (PCs) have landed
solidly in the real world. For example, waveguides within 2‑D PCs
can be engineered to slow light down drastically, potentially
leading to very compact optical delays and buffers for integrated
optics. The bandwidth of such devices, however, is quite narrow.
Toshihiko Baba and his group at Yokohama National University
(Yokohama, Japan) are refining a variety of techniques to widen the
bandwidth (zero-dispersion region) of slow-light photonic-crystal
waveguides. In one device, two PC waveguides, one with no holes and
the other with small holes offset from the lattice by half a period,
are fabricated next to each other. The waveguides have dispersions
opposite to one another; as light propagating down one waveguide
couples into the other, dispersion is canceled over a 9 nm spectral
region and light is slowed to c/50. Baba’s group has also slowed
light to from c/30 to c/40 with a 35 nm bandwidth in a
chirped-structure PC (see Fig. 3); lower speeds and wider bandwidths
will be reached by combining one of these approaches with dynamic
tuning (trapping light without compressing the pulses).
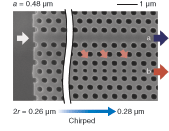
FIGURE 3. Two adjacent photonic-crystal waveguides,
one with no holes (a) and the other with offset holes reduced
in size (b), serve as a coupler that slows light in a
dispersion-free manner. A chirped structure (hole sizes
varying along the length) further refines its
properties. |
Many, if not most, typical 2-D and 3-D PCs have only partial
bandgaps; achieving a complete bandgap is only possible if the
difference in refractive index between the components is high
enough. With a refractive index of 2.2 and a large transmission
window of 0.45 to 5 mm, lithium niobate (LiNbO3) would seem to be the ideal
material for PCs; in addition, its large nonlinear coefficients
would allow harmonic generation and other nonlinear effects.
Guangyong Zhou and Min Gu of the Centre for Micro-Photonics and
CUDOS (an Australia Research Council Centre of Excellence for
ultrahigh-bandwidth devices for optical systems) at Swinburne
University of Technology (Hawthorn, Australia) are creating 3-D PCs
from LiNbO3 using
femtosecond-laser-induced microexplosions to “write” the PC
structure. One problem is the strong spherical aberration induced by
the large index mismatch between the LiNbO3 and its surrounding medium,
which deforms and broadens the focused spot. The researchers
sidestep this problem by writing just above the ablation threshold.
They have fabricated a 16-layer face-centered-cubic PC that
suppresses transmission by up to 30% in its bandgap (see Fig. 4).
Further research includes the use of a liquid-crystal phase
modulator to sharpen the fabrication beam, which will produce PCs
with greater transmission suppression; and studying nonlinear
optical effects in the structure.
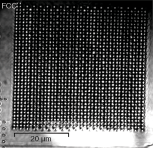
FIGURE 4. A face-centered-cubic (FCC) photonic
crystal is fabricated in lithium niobate with ultrafast-laser
pulses; the pulses cause microexplosions that create voids in
the material. |
Abandoning the concept of crystallinity in photonic
microstructures can have its advantages, as George Barbastathis of
the Massachusetts Institute of Technology (Cambridge, MA) and his
group demonstrates. They have developed “photonic noncrystals”
consisting of, in one theoretical example, many parallel linear
arrays of short vertical rods; the arrays, which have a spacing that
varies as a function of distance from the central axis, form a
planar gradient-index (GRIN) lens (see Fig. 5). Because they are
photolithographically fabricated, the refractive-index profiles of
these GRIN lenses can be very precisely tailored through the spacing
and diameter of the rods; the standard parabolic profile can be
modified to allow tight focusing in very short lengths.
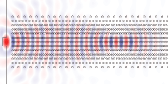
FIGURE 5a. A 2-D “photonic-noncrystal” lens consists
of high-index rods that vary in density from the optical axis
to create an approximately parabolic effective-index
profile. |
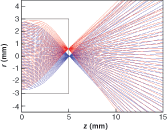
Figure 5b. Optimizing the profile by adding
nonparabolic terms allows the lens to be made very short,
while at the same time reducing the size of the focused spot
(bottom). |
Moving into the domain of nanostructures, researchers at
Northwestern University and OptoNet (both of Evanston, IL) have
developed a 20-µm-long polarization-independent “super GRIN” lens
that can be fabricated on silicon using CMOS-compatible processes.
The lens focuses light (at 1550 nm) in two dimensions: one by a
one-dimensional curve on its entrance face, and the other by
nanolayers of two different optical materials (titanium dioxide and
silicon dioxide) that vary from 25 to 50 nm in thickness,
providing a parabolic index profile. The motivation is to couple
light from a standard single-mode optical fiber directly into
nanosize waveguides such as photonic wells and wires with height and
width on the order of a few hundred nanometers; simulations show a
95.6% coupling efficiency into a 500 nm waveguide.
A fabricated super-GRIN lens has excellent focusing qualities
(see Fig. 6). The lens has a numerical aperture (NA) of 1.5,
although NAs up to 3 are possible in the future with the use of
other material combinations (for example, silicon dioxide and
silicon), says Seng-Tiang Ho, one of the Northwestern researchers.
V-groove super-GRIN arrays have been created, demonstrating their
potential for integration into multifunctional photonic circuits.
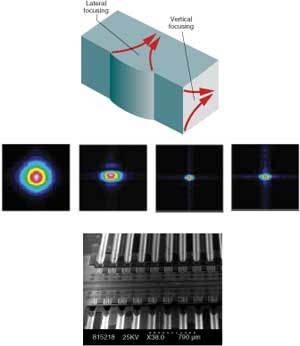
Click here to enlarge
image
FIGURE 6.
Fabrication of a nanolayered GRIN lens (top) is CMOS
compatible. Mode and spot sizes (middle, from left to right)
are compared for single-mode fiber (8 × 8 µm); wedge fiber (3
× 8 µm); a super-GRIN lens (0.9 × 2 µm); and a laser diode (1
× 3.5 µm). Super-GRIN lenses serve to couple light from fibers
in V-groove arrays into planar nanowaveguides
bottom). |
Optical sensing of trace substances is essential in the areas of
security, industrial-process monitoring, science, and others.
Although such techniques often rely on spectroscopy, other
approaches abound. For example, a toroidal optical microcavity
developed by Andrea Armani and Kerry Vahala of the California
Institute of Technology (Pasadena, CA) sensitively measures the
proportion of heavy water (water in which the hydrogen is replaced
by deuterium) in a sample of water.5 The ring is immersed in the
water sample, and light at a 1300 nm wavelength is coupled into the
ring via a tapered optical fiber; the surrounding water slightly
absorbs the evanescent light waves at the surface of the ring,
affecting the cavity’s Q (quality) factor. The absorption
coefficient at 1300 nm for heavy water (0.208 cm‑1) results in a Q of
107; ordinary water
(1.374 cm-1)
produces a Q of 106.
“The concentration of heavy water that occurs in nature is
approximately one in 6400,” says Armani. “Using this technique, it
is possible to detect one heavy-water molecule in 10,000. This is
the first system that is able to cross the threshold and detect
real-time fluctuations in the naturally occurring population.”
Armani notes that the most obvious application is in the development
of nuclear weapons, because heavy water is typically found wherever
someone is trying to control a nuclear chain reaction. However,
there are additional applications in water-supply and environmental
monitoring.
As for spectroscopic detection of common organic substances
(drugs, biological tissue, and industrial chemicals), the mid-IR is
all-important, because it encompasses the vibrational modes of many
molecules. Harnessing the richness of this spectral region has not
been easy, however, due to the lack of an easy-to-use broadly
tunable light source. A quantum-cascade laser developed by Daylight
Solutions (Poway, CA) and integrated into a package a few
centimeters in size has a ±5% tuning range and a center wavelength
that can range from 4.5 to 11.0 µm (see “Broadly tunable QC laser
enters the market,” www.laserfocusworld.com/articles/252457; also,
“Tunable QC laser opens up mid-IR sensing applications,” www.laserfocusworld.com/articles/259939). Combining
this laser with a room-temperature mid-IR detector will result in
small, rugged optical sensors to detect explosives, pollutants, and
even breath-borne substances that indicate disease.
Photonic technology contributes to efficient energy use in a
variety of ways: non-fossil-fuel energy production (photovoltaics);
energy conservation (high-efficiency lighting); and new
oil-exploration techniques (see “Photonics and the energy crisis,”
http://www.laserfocusworld.com/articles/272166)
In photovoltaics, cadmium selenide (CdSe) quantum dots assembled
in titanium dioxide films form solar cells that are tunable in
spectral response (by varying the size of the CdSe dots) and, due to
the fact that high-energy photons can produce multiple charge
carriers in quantum dots, have the potential for a photon-conversion
efficiency of greater than 100% (see “Quantum dots promise
next-generation solar cells,” www.laserfocusworld.com/articles/252486). And, in
the area of organic photovoltaics, which typically are inefficient
and degrade easily, a solar cell based on a new ionic liquid has a
7% efficiency that does not degrade even after 2000 hours of extreme
environmental testing (see “Ionic-liquid solar cell boasts 7%
efficiency,” www.laserfocusworld.com/articles/266359).
One way to boost the efficiency of an inorganic LED is to find a
better way to extract light from the LED chip-which, with its very
high refractive index, tends to hold onto much of its light output
as a result of total internal reflection (TIR) within the chip.
Microstructured, nanostructured, pyramidal, lensed, and other
geometries have been devised for this purpose; now, Wanli Chi and
Nicholas George of the University of Rochester (Rochester, NY) have
created a compact and efficient approach in which an LED is embedded
in a transparent sphere with either a planar reflecting cut or a
small scattering section (see Fig. 7).6 While most rays from an LED
embedded in a dielectric sphere would never escape due to TIR, the
scatterer or planar mirror serve to redirect the rays out, with a
calculated efficiency of 90% or greater for a refractive index of
1.5. For a 300 µm LED die that emits 40% from its front surface and
15% from each of its four sides, the far-field output approaches a
flat-top distribution with a ±10° angular range.
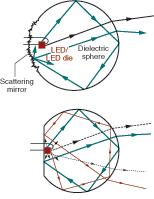
FIGURE 7. Light is extracted at 91% efficiency from
an LED embedded in a transparent sphere that incorporates
either a scatterer (top) or a planar cut serving as a mirror
(bottom). The mirror relies on TIR and does not need a
reflective coating. |
Vertical-cavity lasers developed by Novalux (Sunnyvale, CA) can
be integrated into a large-screen projection-TV display that
consumes a fraction of the electrical power used by a plasma TV (see
“Photonics and the energy crisis,” www.laserfocusworld.com/articles/272166); depending
on electrical rates, the laser TV would pay for itself through
energy savings alone in anywhere from three to eight years. It
appears that this technology is going commercial; Novalux is working
with Arasor (Mountain View, CA), which provides periodically poled
lithium niobate crystals for the TV, in pushing the technology to
the market; plans are for consumer-electronics manufacturers
Mitsubishi and Samsung to launch projection TVs based on Novalux
lasers by December 2007.
The most versatile and productive way to image smaller features
(in the sense of seeing, as in a microscope, rather than printing,
as in photolithography) is to move to shorter wavelengths. While
features as small as 15 nm have been imaged by optical systems that
rely on building-size synchrotrons as extreme-ultraviolet (EUV)
light sources, few researchers have synchrotrons in their labs.
Researchers at Colorado State University (Fort Collins, CO) and
Lawrence Berkeley National Laboratory (Berkeley, CA) have imaged 38
nm features with a Fresnel-zone-plate-based optical system combined
with a tabletop EUV laser. (Also, see “Coherent light sources reach
the extreme ultraviolet,’’ p. 69.)
Joule-level pulses at 5 Hz from a Ti:sapphire laser are focused
onto a solid target to produce a plasma in a nickel-like state,
producing 13 nm light. A 5-mm-diameter condensor zone plate focuses
light onto the object; a 0.1-mm-diameter zone plate with minimum
zone size of 50 nm images onto a back-thinned, EUV-sensitive CCD
with a 2048 × 2048-pixel array. Elbows, dense lines, and complex
test patterns are imaged (see Fig. 8).
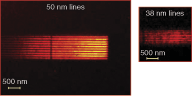
FIGURE 8. Lines and spaces 50 and 38 nm wide are
imaged with a tabletop optical system based on a 13 nm
wavelength. The high modulation of 70% for the 38 nm lines
indicates that even smaller features can be
resolved. |
The industrial-imaging arena has seen much innovation this year.
Princeton Lightwave (Cranbury, NJ) has developed a dual-band camera
that optically combines two very different optical sensors (see
“Infrared cameras vie for multispectral applications,” www.vision-systems.com/articles/274263). One sensor,
for the visible (400 to 900 nm), is a 2048-element linear CCD array;
the other, for IR (1000 to 1700 nm), is a 512-element linear indium
gallium arsenide photodiode array. The alignment places each IR
pixel at every fourth visible pixel for simultaneous aligned
imaging.
In an interesting trend, manufacturers of “smart cameras” (which
contain embedded processors and software) are loading up lots of
third-party imaging software into their cameras, offering systems
integrators multiple software choices when using their cameras (see
“Smart cameras look for smarter uses,” www.vision-systems.com/articles/272328). For
example, a camera by Sony (Tokyo, Japan) can run various x86-based
operating systems, allowing the use of either costly but mature
imaging software on Windows XP, or free applications being developed
for Linux.
The fabrication of aspheric optics heavily relies on
interferometric testing; for surface departures from sphericity of
more than a few waves, null optics are usually required (null optics
change the interferometer’s spherical wavefront to an aspherical one
that matches the desired surface figure of the optic under test).
But null optics themselves are difficult to use. Engineers at QED
Technologies (Rochester, NY) are extending the capabilities of the
company’s subaperture-stitching interferometer (SSI) to handle
optics with higher asphericity, simplifying the measurement
process.7
The SSI combines a standard interferometer by Zygo (Middlefield,
CT) with control software and additional hardware by QED to allow
subaperture measurements to be combined into a full-aperture
interferogram. When used on an asphere, the SSI measures small,
almost spherical segments of the optic and combines them. A mirror
fabricated by SSG-Tinsley (Wilmington, MA) for NASA was tested; the
100-mm-diameter ellipsoid had a base radius of -226 mm and an
aspheric departure of about 12 µm. Measurements with the SSI
agreed well with a separate measurement using null optics (see Fig.
9).
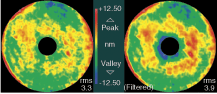
FIGURE 9. A conventional interferometric measurement
(left) of an aspheric mirror uses a retrosphere as the null
optics; a subaperture-stitching measurement of the same mirror
(right) requires no null optics. Both measurements show
similar results. |
The newly commercially introduced blue-light-based
optical-recording media, Blu-ray and HD-DVD disks, can hold anywhere
from 15 to 50 GByte of data; capacities substantially higher than
this will require a change in technology. Researchers at the
University of Miami (Coral Gables, FL) and New Span Opto-Technology
(Miami, FL) may have developed this technology-a holographic
optical-storage technique that retains the conventional bit-oriented
approach and is thus compatible with existing CD and DVD reading and
writing architectures.
Their approach is spectral coding, in which each submicron-size
data site on the disk contains many single-bit holograms, all
written in the same volume and each written by a different
wavelength. The researchers have developed and fabricated a
diffractive/refractive lens with a measured depth of focus of
30 µm to write 0.5 µm spots in many colors, and a “wavelength
combiner” that takes white light and creates dozens of discrete
lines across the visible spectrum, each potentially capable of
writing a different wavelength.
While the technology is still in an early phase, the researchers
have succeeded in writing spectrally coded data with three lasers
(red, green, and blue). They have also fabricated a wavelength
combiner that spectrally splits white light, spectrally modulates it
with a reflective spatial light modulator, and then recombines the
light, resulting in many discrete lines (see Fig. 10). In one
experiment, six gratings were recorded in one spot. While extending
the number of gratings into the dozens (and creating commercially
viable hardware) will require further development, the payoff could
be quite large.
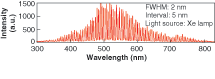
FIGURE 10. A wavelength combiner creates many
spectrally narrow lines from white light; each line can
potentially write its own channel of data on an optical disk
in the form of a submicron-size grating.
|
1. G. Roelkens et al., Optics Express 14(18) (Sept. 4,
2006).
2. H. Rong at al., Optics Express 14(15) (July 242006).
3. J.B. Pendry et al., Science 312, 5781) (June 23, 2006).
4. D. Schurig et al., Science Express Reports, published
online Oct. 19, 2006 [DOI: 10.1126/science.1133628].
5. A.M. Armani and K.J. Vahala, Optics Letters 31(12)
(June 15, 2006). (D2O in H2O)
6. W. Chi and N. George, J. Opt. Soc. Am. A 23(9)
(September 2006).
7. P. Wu et al., FWI2 session presentation, Frontiers in
Optics 2006, the 90th OSA Annual Meeting, Rochester, NY (Oct.
8-12, 2006). Laser Focus World December,
2006 Author(s) : John
Wallace
|